By Dr DF Duvenhage
Introduction
Membrane technology involves using semi-permeable membranes to separate or purify components of a fluid mixture by selectively allowing specific molecules or ions to pass through while blocking others [1]. These membranes act as barriers that separate different contaminants out from the water based mainly on their size. This technology has revolutionized water treatment processes across various industries, particularly mining, where the demand for efficient and effective water management is paramount.
Membrane technologies are integral to modern water treatment processes, offering unique advantages over other methods. In water treatment, the primary goal is to remove problematic contaminants, ensuring the water meets the desired quality standards. This means the pollutants must be transferred from the water to a manageable form, where they can be safely discarded or treated further. Membrane technologies achieve this through selective permeability, allowing specific molecules or ions to pass through the membrane while blocking others. This approach fundamentally differs from other water treatment technologies, such as chemical treatment, coagulation, sedimentation, and biological processes, each with its mechanisms for contaminant removal.
Commercial membrane technologies include microfiltration (MF), ultrafiltration (UF), nanofiltration (NF), and reverse osmosis (RO). They all fit into the broader water treatment landscape by providing precise and efficient filtration capabilities. These technologies are used in various stages of water treatment, from pre-treatment to final polishing, depending on the specific contaminants present and the required water quality. For example, MF and UF are often used to remove suspended solids and pathogens early in the treatment process, protecting downstream equipment and enhancing the effectiveness of subsequent treatment steps [2]. NF and RO, on the other hand, are typically used in later stages to remove dissolved salts, organic molecules, and other fine contaminants, ensuring the highest water quality [3].
Traditional water treatment methods, such as chemical and coagulation, rely on adding chemicals to the water to neutralize or precipitate contaminants. In coagulation, chemicals like alum are added to form flocs, which aggregate and settle out of the water, taking pollutants with them. While effective, this process generates sludge that must be managed and disposed of, presenting additional environmental challenges.
Sedimentation and filtration are mechanical processes that physically separate contaminants based on their size and weight, or density [4]. Sedimentation allows heavier particles to settle to the bottom of a treatment tank, while filtration uses media such as sand or activated carbon to trap particles as water passes through. Biological treatment methods, such as activated sludge processes, use microorganisms to break down organic contaminants [5]. These methods are highly effective for specific contaminants but may not be suitable for others, such as dissolved salts or heavy metals.
Unlike these methods, membrane technologies provide a targeted approach to contaminant removal. These technologies can selectively remove contaminants based on their size, charge, or chemical affinity by utilizing semi-permeable membranes with specific pore sizes and properties. This allows for precise control over the treatment process and removes a wide range of contaminants with a single technology.
Furthermore, membrane technologies typically produce a concentrated waste stream known as brine, which contains the removed contaminants. This concentrated stream can be easier to manage and dispose of than the large volumes of sludge generated by coagulation and sedimentation processes. For example, in reverse osmosis, the brine contains the salts and impurities removed from the feed water, which can then be further treated or disposed of into an appropriate effluent stream or evaporation ponds [6].
The concept of membrane filtration dates back to the mid-18th century when scientists first observed selective permeability in natural membranes. However, synthetic membranes weren’t developed until the early 20th century. In 1907, Bechhold pioneered the creation of synthetic membranes using nitrocellulose, laying the groundwork for future advancements in membrane technology [7]. These early membranes were primarily used for laboratory applications and the filtration of colloidal solutions.
The commercialization of microfiltration and ultrafiltration began in the 1960s and 1970s. The dairy industry initially used these technologies to concentrate milk and whey proteins [8]. Companies developed robust polymeric membranes, which enabled broader industrial applications, including water treatment, food processing, and biotechnology [9]. During this period, significant research focused on improving membrane materials and configurations. Introducing asymmetric membranes featuring a dense selective layer on porous support enhanced the performance and durability of MF and UF membranes [10]. These advancements paved the way for the widespread adoption of microfiltration and ultrafiltration in various industries.
Nanofiltration emerged in the late 1980s and early 1990s as a technology designed to bridge the gap between ultrafiltration and reverse osmosis [11]. NF membranes can selectively remove divalent ions and larger organic molecules while allowing monovalent ions to pass through. This selective permeability made nanofiltration ideal for applications such as water softening, removal of organic contaminants, and partial desalination. The development of thin-film composite (TFC) membranes was a critical milestone for nanofiltration. TFC membranes, consisting of a polyamide selective layer on a polysulfone support, offered improved flux and rejection characteristics. These membranes became the industry standard for NF applications and continue to dominate the market today [12].
Reverse osmosis’s roots lie in the 1950s, when researchers began exploring methods to desalinate seawater. The development of practical RO membranes can be attributed to the work of Loeb and Sourirajan, who in 1960 developed the first asymmetric cellulose acetate membranes capable of achieving significant salt rejection [13]. This breakthrough marked the beginning of modern reverse osmosis technology.
The 1970s and 1980s saw rapid advancements in RO membrane technology, driven by the demand for large-scale desalination and industrial water treatment. The introduction of polyamide thin-film composite membranes in the late 1970s revolutionized the industry, offering superior performance and chemical resistance compared to cellulose acetate membranes [12]. These TFC membranes remain the gold standard in reverse osmosis applications.
In recent decades, membrane technologies have continued to evolve, driven by the need for more efficient, durable, and cost-effective solutions. Advances in membrane materials, such as the development of fouling-resistant and high-flux membranes, have significantly improved the performance and lifespan of MF, UF, NF, and RO systems [14]. Additionally, membrane module design and process optimization innovations have enhanced these technologies’ scalability and energy efficiency.
How membranes work
Microfiltration (MF)
Filtration is the process of separating suspended solid matter from a liquid. Reverse osmosis (RO) and nanofiltration (NF) membranes pass and reject molecules primarily based on charge characteristics. In terms of ultrafiltration (UF) and microfiltration (MF), however, the membrane rejects particles based on size. Microfiltration (MF) operates based on the principle of size exclusion. MF membranes have pore sizes ranging from 0.1 to 10 micrometers, which enables them to effectively remove particles, bacteria, and some protozoa from water [15]. The mechanism of MF can be understood through the concepts of sieving and surface filtration, as illustrated in Figure 1.

Figure 1: Membrane filtration diagram; https://nordicmembrane.com/membrane_technology
The size exclusion principle dictates that particles larger than the membrane’s pore size are retained on the surface or within the pores while smaller particles and water molecules pass through. This process is akin to a sieve where the membrane acts as a barrier, allowing only particles smaller than the pore size to permeate. MF’s effectiveness in removing contaminants largely depends on the uniformity and size distribution of the membrane pores.
In surface filtration, particles are captured on the surface of the membrane, forming a filter cake. As the filter cake builds up, it can enhance the filtration efficiency by trapping even smaller particles [16]. However, this also leads to increased resistance to flow, requiring periodic cleaning or backwashing to maintain performance. The membrane surface exhibits a range of pore sizes, so particles close to the size of these pores may partially enter and obstruct them, leading to a decrease in flux. Therefore, maintaining an effective cleaning regimen is crucial to prevent pore-clogging particles from accumulating on the membrane surface. If many pores become blocked, the membrane may become irreversibly clogged.
Microfiltration is widely used in various industries, including water and wastewater treatment, food and beverage processing, pharmaceuticals, and biotechnology. In water treatment, MF is typically used as a pre-treatment step to remove suspended solids, bacteria, and other large particulates before subsequent filtration processes like ultrafiltration or reverse osmosis.
Ultrafiltration (UF)
Like most conventional filtration methods, sand filters and media filtration rely on consistent raw water quality to produce quality effluent, which can be challenging to maintain. These filters also do not provide an absolute barrier. Traditional media filters usually remove particles down to approximately 5 microns. Ultrafiltration (UF) membranes, with pore sizes between 10-100 nanometres, can remove viruses, colloids, and smaller pathogens from water. These membranes are often used in conjunction with other filtration systems to provide a comprehensive barrier against a broad spectrum of contaminants [17]. Ultrafiltration is particularly valuable in treating process water and wastewater in mining, ensuring that recycled water meets stringent quality standards and can be safely reused or discharged. The UF process operates similarly to MF but requires slightly higher pressure due to the finer membrane structure. This process removes smaller contaminants, allowing water and low molecular weight solutes to pass through.
Ultrafiltration (UF) membranes can be categorized based on their molecular weight cut-off (MWCO) in Daltons (1 Dalton equals 1 atomic mass unit) or by pore size in microns. These membranes typically fall within a range of about 1,000 to 500,000 Daltons. However, for more open membranes with MWCOs greater than 100,000 Daltons, classification by pore size is more common [15].
UF Membranes are asymmetrical, with a thin, skin-like material on the surface of the membrane. The layers below this skin typically consist of voids supporting the skin layer on top. Since rejection occurs at the membrane surface, particles or macromolecules larger than the nominal MWCO do not penetrate the voids or the main body of the membrane. Consequently, these membranes are prone to fouling.
MF and UF membranes separate relatively large molecules such as proteins, starches, gums, clays, paints, pigments, and suspended solids; the osmotic pressures involved in these processes are very low. Consequently, UF and MF systems operate at significantly lower pressures than reverse osmosis or nanofiltration systems.
Nanofiltration (NF)
Nanofiltration (NF) was developed after reverse osmosis (RO) as a more permeable alternative to bridge the gap between RO, which rejects nearly all salt ions and most uncharged organic solutes, and UF, which permits the passage of ionic species while retaining uncharged solutes with molecular weights as small as several thousand Daltons. The need for new applications prompted the development of NF membranes, as neither RO nor UF membranes could provide the required separations. The earliest documented application was water softening in Florida in the late 1970s, with the first documented process of NF membrane being commercialized for the purpose of desalting a small food-grade dye in 1983 [18].
Nanofiltration (NF) membranes, characterized by pore sizes in the range of 1-10 nanometres, are designed to remove divalent and larger monovalent ions, such as calcium and magnesium, and organic molecules. This selective ion removal capability makes nanofiltration ideal for applications requiring water softening and removal of specific contaminants while retaining beneficial minerals. In the mining industry, nanofiltration helps concentrate valuable minerals and reduce the presence of undesirable ions that can interfere with extraction processes. NF membranes operate at higher pressures than UF, selectively allowing water and certain ions to pass while rejecting larger ions and molecules.
Similar to reverse osmosis, NF is a pressure-driven membrane filtration process that employs a semi-permeable membrane and cross-flow filtration to divide a feed into a purified permeate stream and a concentrated stream, which contains a high concentration of impurities from the raw water. NF operates at lower pressures than RO and features a slightly more open membrane structure that primarily allows monovalent ions to pass through while rejecting most divalent ions. This property is particularly advantageous in water softening applications, where NF technology is used to reduce hardness caused by calcium and magnesium, as well as to remove organics, color, bacteria, trihalomethane (THM) precursors, and other contaminants from the raw water supply.
NF is also relevant in various process applications, such as divalent ion concentration, dextrose purification, and food and dairy industries, where piperazine NF membranes are employed to allow 50 to 90% passage of monovalent ions while rejecting the majority of divalent ions. Although RO is essential for seawater desalination and brackish water treatment due to its capability to handle very high levels of dissolved solids (TDS), many water supplies do not require the nearly complete salt removal that RO provides. NF membranes offer partial demineralization, removing between 10 to 90% of dissolved salts compared to over 99% removal by RO.
Reverse osmosis (RO)
Reverse osmosis (RO) membranes have the smallest pore sizes, typically less than 1 nanometre, and can remove nearly all dissolved salts, organic molecules, and pathogens from water. The high-pressure operation of reverse osmosis systems forces water through the membrane, leaving contaminants behind. RO is extensively used in desalination plants to produce potable water from seawater and in mining operations to provide high-purity water for sensitive processes and equipment. The process involves applying high pressure to the feed water, forcing it through the semi-permeable membrane while leaving the contaminants behind. This results in the separation of clean water (permeate) and a concentrated waste stream (brine).
A membrane acts as a semi-permeable barrier that permits certain molecules to pass through while rejecting others. Osmosis refers to the natural movement of pure water across a membrane. In this process, a semi-permeable membrane is positioned between two compartments in a tank; one side contains high-purity water with low salt content, and the other side has water with lower purity and higher salt content, as shown in Figure 2. If the membrane is permeable only to water and not to dissolved salts, the system will naturally move toward equilibrium, resulting in both compartments having equal salt concentrations. Consequently, pure water from the less concentrated side flows through the membrane, which retains the salts, towards the more concentrated side [18].
Although reverse osmosis (RO) and nanofiltration (NF) can also remove particulate matter, their non-porous, semi-permeable membranes are susceptible to rapid fouling when exposed to high levels of particulates. In these processes, when pressure exceeding the natural osmotic gradient is applied to the feed side of the membrane, water is pushed through the membrane’s molecular structure, while the dissolved solids (solutes) are mostly retained. Although solutes can also pass through the semi-permeable membranes, their diffusion rate is much slower than water’s. As a result, the permeate, or the water that emerges from the membrane, has a lower concentration of dissolved solids than the feed water entering the system.
Although RO and NF can also remove particulate matter, their non-porous, semi-permeable membranes are susceptible to rapid fouling when exposed to high levels of particulates. In these processes, when pressure exceeding the natural osmotic gradient is applied to the feed side of the membrane, water is pushed through the membrane’s molecular structure, while the dissolved solids (solutes) are mostly retained. Although solutes can also pass through semi-permeable membranes, their diffusion rate is much slower than water’s. As a result, the permeate, or the water that emerges from the membrane, has a lower concentration of dissolved solids than the feed water entering the system.
The energy required to drive the feedwater through the membrane is influenced by the membrane’s material and thickness, as well as the osmotic pressure of the feed solution. Osmotic pressure is the force exerted by the natural process where water moves from a dilute solution (with fewer dissolved solids) through a semi-permeable membrane to a more concentrated solution (with higher dissolved solids). Therefore, hydraulic pressure is needed to overcome both the membrane’s physical resistance and the system’s osmotic pressure. This pressure counteracts the natural osmotic gradient to produce less saline water from a more concentrated source, which is why reverse osmosis is termed [19].
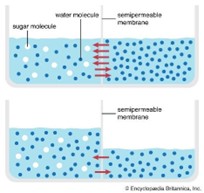
Figure 2: Th osmotic process – https://external-content.duckduckgo.com/iu/?u=https%3A%2F%2Fcdn.britannica.com%2F81%2F62981-050-22A37BC3%2Fprinciple-permeation-water-diffusion-sugar-differences-membrane.jpg&f=1&nofb=1&ipt=344839451248e502faade8712c8577624bde71ce3
Reverse osmosis (RO) is highly effective at rejecting over 99% of dissolved salts (ions), particles, colloids, sugars, organics, pesticides/herbicides, and endotoxins/pyrogens from feed water. RO membranes achieve this by rejecting contaminants based on their size and charge. Contaminants with a molecular weight greater than 100 Da are likely to be rejected by an RO membrane. Additionally, contaminants with higher ionic charges are less likely to pass through; for example, RO membranes efficiently reject magnesium and sulfate ions, which carry 2+ and 2- charges (divalent), whereas sodium and chloride ions, with 1+ and 1- charges (monovalent), are less easily rejected.
RO is widely used for treating brackish, surface, and groundwater in both large and small-scale applications. Industries that utilize RO-treated water include municipal drinking water, pharmaceuticals, boiler feed water, food and beverage, metal finishing, and semiconductor manufacturing. In some processes, RO membranes are used to concentrate proteins and sugars, where the concentrate is the valuable product and the permeate is the byproduct.
Overall, the effectiveness of membrane technology in contaminant removal is attributed to the precise engineering of membrane materials and structures, which are tailored to target specific types of contaminants. Integrating membrane systems into water treatment processes enhances the quality of treated water and contributes to the sustainability of industrial operations by enabling the recycling and reuse of water resources. As advancements in membrane technology continue to evolve, their role in ensuring water security and environmental protection becomes increasingly vital.
Membrane technologies as part of a treatment system
- Membrane pretreatment considerations
- Membrane technology peripherals (pumps, instrumentation, etc)
- Clean in place system (CIP)
- Brine management
- Permeate remineralisation
Membrane technologies are essential in various industrial applications, especially in water and wastewater treatment, due to their efficiency in separating contaminants from liquids. Understanding the equipment specifications for microfiltration (MF), ultrafiltration (UF), and reverse osmosis (RO) technologies is crucial for effective application. Microfiltration systems use membranes with pore sizes typically ranging from 0.1 to 10 micrometers to remove suspended solids, bacteria, and other particulates. They operate at low pressures (1 to 3 bar) and have flux rates between 50 and 200 liters per square meter per hour (LMH). Common membrane materials include polymeric substances like polyvinylidene fluoride (PVDF) and ceramics, with configurations such as hollow fiber, tubular, flat sheet, or spiral wound. Regular maintenance involves backwashing and chemical cleaning to maintain permeability.

Figure 3: Membrane equipment system diagram; https://www.metawater.co.jp/eng/solution/product/water/membrane_clarify/
A standard membrane treatment system typically comprises three distinct subsystems: pretreatment, the membrane process, and posttreatment. As depicted in Figure 3, the system includes an influent stream (feed) and two effluent streams (permeate and concentrate). Water sources fed into RO and NF systems generally require specific pretreatment. This often involves adding acid, scale inhibitors, or both to prevent the formation of insoluble salts as the concentration of rejected ions increases, followed by 5- to 20-micron cartridge filtration to protect the RO/NF membranes from particulate fouling.
Additional pretreatment steps might be needed before the standard cartridge filters for water sources with higher fouling potential, such as surface waters. Posttreatment or wastewater treatment can include various processes typical of conventional drinking water treatment, such as aeration, degasification, pH adjustment, addition of corrosion control chemicals, fluoridation, and disinfection. To successfully design and operate an RO or NF treatment plant, it is crucial to thoroughly understand the characteristics of the source water and the quality goals for the treated water, as these factors dictate the appropriate combination of pretreatment and posttreatment techniques required.
Pre-treatment
Extensive pre-treatment is important for all membrane treatments. However, they become more critical as one progresses from MF to RO systems to prevent membrane fouling and scaling. This often includes softening, anti-scalant dosing, pH adjustment, and multiple stages of filtration (such as microfiltration or ultrafiltration).
Softening is a crucial pre-treatment step for membrane filtration technologies, particularly in systems utilizing reverse osmosis (RO) and nanofiltration (NF). The primary objective of softening is to remove hardness-causing minerals, such as calcium and magnesium ions, which can lead to scaling on membrane surfaces. Scaling can significantly impair membrane performance by reducing flux rates and increasing operational costs. Softening typically involves ion exchange processes, where hard water is passed through a bed of ion exchange resin that replaces calcium and magnesium ions with sodium ions. This process can prevent the formation of scale deposits by reducing the concentration of hardness ions in the feed water. In some cases, lime softening or precipitation methods are also used to lower the hardness levels before the water reaches the membrane system. Effective softening ensures that the membrane operates efficiently and has a longer service life, reducing the frequency and cost of maintenance.
Anti-scalant dosing is another essential pre-treatment step, particularly for RO systems, to prevent the formation of scale deposits on membrane surfaces. Anti-scalants are chemical additives that interfere with the crystallization of scale-forming minerals such as calcium carbonate, calcium sulfate, and barium sulfate. By preventing these minerals from precipitating out of the solution, anti-scalants help maintain the membrane’s permeability and overall performance. The choice and dosage of anti-scalants are determined based on the water’s composition and the specific scaling risks associated with it. Regular monitoring of the feed water and membrane performance ensures that the correct amount of anti-scalant is used, which is vital for effective scale control. Proper dosing of anti-scalants minimizes the risk of membrane fouling and helps maintain the operational efficiency of the filtration system.
pH adjustment is a critical pre-treatment step for optimizing membrane performance and longevity. The pH of the feed water can significantly influence the solubility of various contaminants and the potential for scaling and fouling. For instance, water with a high pH can lead to the precipitation of scale-forming minerals, while low pH can cause membrane degradation or corrosion. Adjusting the pH of the feed water involves either adding acidic or alkaline substances to bring the pH to an optimal range that enhances membrane performance and minimizes fouling and scaling risks. This process is typically carried out using automated dosing systems that add precise amounts of chemicals such as sulfuric acid or sodium hydroxide. Maintaining the feed water within the recommended pH range ensures that the membrane operates efficiently and has a prolonged service life, contributing to the overall effectiveness of the water treatment system.
Multiple stages of filtration, such as microfiltration (MF) or ultrafiltration (UF), are often employed as a pre-treatment step to remove particulate matter and larger contaminants from the feed water before it reaches the RO or NF membranes. Microfiltration and ultrafiltration use membranes with varying pore sizes to separate particles based on size. Microfiltration typically removes particles larger than 0.1 microns, including suspended solids, algae, and some bacteria, while ultrafiltration can handle particles down to about 0.01 microns, including smaller bacteria and some viruses. By implementing these filtration stages, the feed water is pre-conditioned to remove a significant portion of the particulate load, thereby reducing the risk of fouling and clogging in the downstream RO or NF membranes. This staged approach not only enhances the overall efficiency of the filtration process but also helps in maintaining the integrity and performance of the more sensitive membrane technologies used in the final treatment stages.
Pumps
In membrane treatment technologies, different types of pumps are utilized to address the specific requirements of each filtration process, including microfiltration (MF), ultrafiltration (UF), nanofiltration (NF), and reverse osmosis (RO). Each type of pump is selected based on its capability to handle varying pressures, flow rates, and the physical characteristics of the feed water.
Microfiltration (MF) and Ultrafiltration (UF) Pumps
Microfiltration and ultrafiltration typically use centrifugal pumps. These pumps suit the relatively low pressures required for MF and UF processes. For MF systems, pumps generally operate in the range of 2 to 10 kW, with flow rates ranging from 10 to 100 cubic meters per hour (m³/h) and operating heads of approximately 5 to 20 meters. Ultrafiltration systems often require slightly more powerful pumps, operating between 5 to 15 kW, with flow rates from 20 to 150 m³/h and operating heads of 10 to 30 meters [20]. These pumps handle the lower pressures and higher flow rates typical of MF and UF processes, ensuring effective filtration and consistent performance.
Nanofiltration (NF) Pumps
Nanofiltration processes require higher pressure pumps than MF and UF due to the membrane’s finer filtration capability. NF pumps are typically positive displacement pumps, such as diaphragm or rotary vane pumps, that operate in the range of 15 to 30 kW. They provide flow rates between 10 to 50 m³/h and handle operating heads of approximately 5 to 20 bar [21]. These pumps need to generate sufficient pressure to overcome the osmotic pressure and drive water through the NF membranes, which have smaller pore sizes than UF membranes and require higher operational pressures.
Reverse Osmosis (RO) Pumps
Reverse osmosis systems demand the highest pressures among membrane technologies, necessitating high-pressure pumps. These pumps are typically multi-stage centrifugal pumps or high-pressure positive displacement pumps designed to operate in the range of 50 to 200 kW, with flow rates from 5 to 50 m³/h and operating heads of up to 80 to 120 Bar in the case of ultra high pressure systems [22]. Due to the dense and selective nature of RO membranes, which reject nearly all dissolved salts and contaminants, significant pressure is required to force water through the membranes. Energy recovery devices, such as pressure exchangers or turbochargers, are often integrated into RO systems to enhance energy efficiency by recovering and reusing a portion of the energy from the high-pressure concentrate stream [23]. These devices help reduce the overall energy consumption of the RO system, making the process more cost-effective.
In summary, the choice of pump type and specifications for membrane treatment technologies is crucial for optimizing system performance and efficiency. Each type of pump is selected based on the specific pressure and flow rate requirements of the filtration process, ensuring that the system operates effectively and efficiently across various membrane technologies.
Clean-in-Place (CIP) Equipment
Clean-in-Place (CIP) is an essential procedure for maintaining the efficiency and longevity of membrane systems, particularly in reverse osmosis (RO) setups. The CIP process allows for the internal cleaning of membranes without requiring disassembly of the water treatment system. This in-situ cleaning method helps manage and remove foulants that accumulate on membrane surfaces during regular operations, which can otherwise degrade performance and reduce membrane lifespan.
The primary purpose of CIP is to restore membrane performance by removing contaminants that impair filtration efficiency. Regular cleaning through CIP can lower operating pressures, enhance water quality, and extend the operational life of the membranes. Effective CIP procedures help optimize membrane performance by addressing fouling issues before they lead to significant system downtime or membrane damage. By following manufacturer guidelines and adjusting cleaning protocols based on performance data, facilities can ensure that their membrane systems operate at peak efficiency.
Designing an effective CIP system involves several critical considerations to ensure optimal cleaning and system performance. The CIP system is typically integrated with the RO system using either flexible hoses or fixed piping. Large RO systems often use fixed piping, as the high flow rates and pressures involved make flexible hoses less practical and potentially unsafe [24]. The materials used for CIP systems must be robust enough to withstand a wide pH range (1-13) and temperatures up to 50°C (122°F). Cleaning is performed at low pressures to minimize permeate production and avoid redepositing dirt on the membrane. High cross-flow velocities are crucial to effectively remove foulants from the membrane surface.
The choice of cleaning chemicals depends on the types of foulants present on the membrane. High pH solutions, such as those containing sodium hydroxide, are typically used to address biological matter, while low pH cleaners, like nitric acid, are effective for removing mineral scale https://www.royalfilters.com/articles/choosing-the-right-chemicals-for-cip. In many cases, a two-stage cleaning process that involves first using a low pH cleaner followed by a high pH cleaner proves most effective. Formulated cleaning chemicals, while more specialized and sometimes costly, offer advantages such as extended system run times, reduced cleaning frequency, and increased longevity of RO system elements.
The CIP system should be designed to handle both the initial cleaning phase, where concentrate and permeate are sent to drain, and the subsequent phase, where they are recycled back to the cleaning tank. Proper sizing of the cleaning pump is also crucial; it should be capable of handling the recommended flows and pressures while accounting for pressure losses in the system https://www.pumpsandsystems.com/how-choose-right-pump-ro-systems. The pump should be constructed from materials like 316 stainless steel or nonmetallic composites to ensure durability and resistance to the cleaning chemicals used.
In conclusion, an effective CIP procedure is integral to the successful operation and maintenance of membrane systems. By carefully designing the CIP system, selecting appropriate cleaning chemicals, and adhering to manufacturer guidelines, facilities can ensure their membrane systems operate efficiently, with reduced downtime and extended membrane life.
Instrumentation and Controls
Membrane systems require sophisticated control systems to monitor pressure, flow rates, conductivity, pH, and temperature. Automated systems help manage operational parameters and trigger maintenance actions. These include pressure sensors, flow meters, and turbidity sensors that provide real-time data on the system’s performance. Automated control systems use this data to optimize operational parameters and trigger maintenance actions when necessary.
While the fundamental principles of monitoring and control remain consistent across these technologies, the specific requirements and parameters can vary significantly depending on the membrane type and its application.
MF systems generally use pressure sensors, flow meters, and turbidity sensors. Pressure sensors monitor the pressure across the membrane to detect fouling or blockages [25]. Flow meters track the permeate volume to ensure the system operates within its designed capacity. Turbidity sensors measure the cloudiness of the filtered water, indicating the presence of suspended solids that can affect performance [26]. Automated control systems use this data to adjust operational parameters and trigger maintenance as needed.
UF systems require similar monitoring equipment to MF, including pressure sensors, flow meters, and turbidity sensors. However, UF membranes handle finer contaminants compared to MF, so maintaining accurate pressure readings and monitoring fouling is even more critical [27]. Flow meters and pressure sensors help manage the operation within optimal ranges, while turbidity sensors ensure that the filtration process is effectively removing particulates.
NF systems operate at higher pressures than MF and UF, making pressure sensors crucial for monitoring and managing these higher pressures [28]. Flow meters are essential to track the permeate and concentrate streams, while conductivity sensors are used to measure the concentration of dissolved salts and other solutes. NF systems also often incorporate pH sensors to manage the chemical environment of the feed water, which can impact membrane performance.
RO systems are the most complex and require precise control of pressure, flow rates, conductivity, pH, and temperature [29]. High-pressure pumps are used to force water through the dense RO membranes, and pressure sensors monitor this force to ensure it remains within optimal ranges. Conductivity sensors are crucial for assessing the efficiency of salt rejection, while pH sensors help prevent membrane damage by monitoring water acidity. Temperature sensors provide data on the operational environment, which affects membrane performance and longevity.
While the core C&I components, such as pressure sensors, flow meters, and automated control systems, are common across all membrane technologies, the specific requirements vary based on the membrane type and its operational conditions. For example, RO systems necessitate more advanced pressure and conductivity monitoring due to their high-pressure operation and need for precise salt rejection. In contrast, UF and MF systems, dealing with less stringent pressures and larger particles, require less complex monitoring setups but still benefit from real-time data to optimize performance [30].
Membrane Applications in Mining Operations (1000)
The mining industry faces complex challenges encompass resource utilization, environmental stewardship, and operational efficiency. As mining operations continue to scale and evolve, the need for sustainable and efficient technologies has become more pressing. Membrane technology has emerged as a crucial solution in addressing these challenges, offering innovative methods for water purification, particle separation, metal recovery, gas separation, and solvent management. This article explores how membrane technologies are applied in mining operations to enhance sustainability and efficiency, focusing on raw water treatment, water reclamation, water conditioning, and the management of process water, tailings, and effluent.
Raw Water Treatment
Mining operations typically require large volumes of water, which can be sourced from surface water, groundwater, or other alternative sources. The quality of this raw water can significantly impact the efficiency and effectiveness of mineral processing. Membrane technologies, such as MF and UF, are employed to treat raw water by removing suspended solids, colloidal particles, and microorganisms.
MF and UF membranes, with pore sizes ranging from 0.1 to 10 microns, and 1-100 nanometers, respectively, effectively remove larger particles and microorganisms, ensuring that the water entering the processing stages is clean and free from contaminants that could impede downstream processes [31]. Ultrafiltration, with smaller pore sizes, further polishes the water by removing finer particles and dissolved organic compounds. This pre-treatment improves the quality of process water and enhances mineral extraction and processing efficiency.
Water Reclamation
Water reclamation is critical to modern mining operations, particularly in arid regions where water resources are scarce. Effective water reclamation involves treating various effluent streams generated during mining to make them suitable for reuse. Membrane technologies play a vital role in this process, offering solutions for treating both process water and tailings water.
In the context of water reclamation, NF and RO are commonly employed. Nanofiltration membranes, with pore sizes ranging from 1 to 10 nanometers, effectively remove divalent ions and organic molecules from water. Reverse osmosis, with even smaller pore sizes, is used to achieve a higher level of purification by removing nearly all dissolved salts, organic compounds, and other contaminants. By employing these technologies, mining operations can reclaim water from effluent streams and use it for various purposes, including ore processing and site reclamation, thereby reducing the overall water footprint.
Water Conditioning
Water conditioning is essential in mining operations to ensure that the water used in different stages of mineral processing meets specific quality requirements. For instance, water used in boilers, cooling towers, and gland service water must be conditioned to prevent scaling, corrosion, and other issues affecting equipment performance and longevity.
Membrane technologies, particularly softening and filtration processes, are employed to treat water for these specific uses. Softening involves removing hardness-causing ions, such as calcium and magnesium, using ion exchange membranes or other treatment methods [32]. This process is crucial for preventing scale buildup in boilers and cooling systems. Additionally, pre-treatment steps such as microfiltration or ultrafiltration can be used to remove particulate matter and colloidal substances that might otherwise cause issues in sensitive applications.
Process Water Treatment
In mineral processing, water is used extensively for ore washing, grinding, and flotation. The quality of process water directly influences the efficiency of these operations. Membrane technologies, such as microfiltration and ultrafiltration, are employed to treat process water by removing suspended solids and colloidal particles. By ensuring that the water used in mineral extraction is free from contaminants, these technologies contribute to improved recovery rates and operational efficiency.
Tailings Water Treatment
Tailings, which are the by-products of mining operations, contain a mixture of water, fine mineral particles, and residual chemicals. Effective management of tailings water is crucial to prevent environmental contamination. Nanofiltration and reverse osmosis are applied to treat tailings water by removing harmful ions and contaminants. This treatment facilitates the safe discharge of water or its reuse within the mining operation, thereby reducing the overall water footprint and minimizing environmental impact.
Effluent Treatment
Effluent from mining operations often contains heavy metals, salts, and other contaminants that must be removed before discharge into the environment. Reverse osmosis membranes are particularly effective in treating mining effluent, ensuring that the discharged water meets regulatory standards. The high removal efficiency of RO systems helps protect local water bodies and ecosystems from contamination, contributing to the sustainability of mining operations.
Conclusion
Membrane technologies have revolutionized water treatment in the mining sector by offering high efficiency in contaminant removal, minimal chemical usage, and reduced waste generation. Their modularity and scalability make them suitable for various applications, from small-scale systems to large industrial operations. These technologies provide versatile solutions for raw water treatment, reclamation, and conditioning. Their ability to achieve high levels of purification while integrating seamlessly with other treatment processes underscores their value in modern water management strategies.
Despite these advantages, membrane technologies face significant challenges, particularly in terms of capital expenditure (CAPEX) and operational expenditure (OPEX). The initial investment for membrane systems can be high, reflecting the costs of purchasing and installing advanced filtration technologies. For the South African mining sector, where capital can be constrained by fluctuating commodity prices, these initial costs can be a barrier. Furthermore, the complexity of these systems and the need for specialized infrastructure contribute to the substantial CAPEX.
Operational costs also pose challenges. Membrane systems require significant energy inputs, especially for high-pressure processes like RO. In South Africa, where energy costs are volatile and the electricity grid is under strain, these fluctuations can impact the financial viability of membrane technologies. Additionally, membrane maintenance and cleaning add to OPEX, with the need for frequent clean-in-place (CIP) procedures and specialized cleaning chemicals.
Economic cycles related to commodity prices significantly influence the feasibility of investing in and maintaining membrane technologies. During periods of high commodity prices, mining companies may be more inclined to invest in advanced technologies due to increased revenue and profitability. However, during downturns, financial pressures to reduce both CAPEX and OPEX can lead to the postponement or reevaluation of such investments. This economic variability, along with factors like exchange rate fluctuations and inflation, can affect the overall cost-effectiveness of membrane systems.
Despite these limitations, membrane technologies offer several key advantages. They achieve high levels of contaminant removal with minimal chemical use and waste generation, making them environmentally friendly and efficient. Their ability to handle concentrated waste streams and integrate with other treatment processes, such as advanced oxidation, enhances their effectiveness in complex water treatment scenarios. Continuous advancements in membrane materials and fabrication techniques are also improving performance and cost-effectiveness. Innovations like fouling-resistant membranes, energy-efficient processes, and hybrid systems are making these technologies more sustainable and efficient.
In the mining sector, the integration of membrane technologies represents a paradigm shift towards more sustainable and efficient mineral extraction processes. By providing tailored solutions for water management, particle separation, metal recovery, and solvent management, these technologies help mining operations meet environmental regulations and reduce their water footprint. As the industry evolves, ongoing research and development will be crucial in optimizing membrane-based processes and expanding their adoption across various applications.
The application of membrane technologies in the South African mining sector highlights a balance between their substantial benefits and the financial challenges they present. While high CAPEX and OPEX, influenced by economic fluctuations, pose limitations, the advantages of these technologies in improving water quality, operational efficiency, and environmental sustainability are significant. As the mining industry faces increasing pressure to manage resources responsibly and meet regulatory standards, the continued advancement and adoption of membrane technologies will be essential in driving a more sustainable and efficient future for mineral extraction.
Bibliography
[1] | [Online]. Available: https://www.mdpi.com/2077-0375/14/7/148. |
[2] | [Online]. Available: https://appliedmembranes.com/back-to-basics-about-ultrafiltration-uf.html. |
[3] | [Online]. Available: https://www.mdpi.com/2077-0375/8/2/17. |
[4] | [Online]. Available: https://www.wef.org/globalassets/assets-wef/direct-download-library/public/03—resources/wsec-2017-fs-022-liquid-stream-fundamentals–clarification-sedimentation_final.pdf. |
[5] | [Online]. Available: https://www.ncbi.nlm.nih.gov/pmc/articles/PMC10376923/. |
[6] | [Online]. Available: https://www.ncbi.nlm.nih.gov/pmc/articles/PMC7281250/. |
[7] | [Online]. Available: https://catalogimages.wiley.com/images/db/pdf/0470854456.01.pdf. |
[8] | [Online]. Available: https://www.sciencedirect.com/science/article/abs/pii/S0011916411004681. |
[9] | [Online]. Available: https://link.springer.com/article/10.1007/s10311-019-00895-9. |
[10] | [Online]. Available: https://www.sciencedirect.com/science/article/abs/pii/S2213343724007589. |
[11] | [Online]. Available: https://www.sciencedirect.com/science/article/abs/pii/S2214714417302787. |
[12] | [Online]. Available: https://www.ncbi.nlm.nih.gov/pmc/articles/PMC8593864/. |
[13] | [Online]. Available: https://www.fgwater.com/static/upload/file/20230615/1686810204956844.pdf. |
[14] | [Online]. Available: https://www.sciencedirect.com/science/article/abs/pii/S1385894724025166. |
[15] | [Online]. Available: https://water-membrane-solutions.mann-hummel.com/content/dam/lse-wfs/product-related-assets/manuals-guides/TB-024-Ultrafiltration-Microfiltration-How-Membranes-Work.pdf. |
[16] | [Online]. Available: https://www.sciencedirect.com/science/article/abs/pii/S0376738819327826. |
[17] | [Online]. Available: https://www.dupont.com/water/technologies/ultrafiltration-uf.html. |
[18] | [Online]. Available: https://water-membrane-solutions.mann-hummel.com/content/dam/water-membrane-solutions/download/bulletins—guides/microdyn/microdyn-reverse-osmosis-nanofiltration-how-membranes-work-technical-bulletin.pdf/_jcr_content/renditions/original./microdyn-reverse. |
[19] | [Online]. Available: https://www.awwa.org/portals/0/files/publications/documents/m46lookinside.pdf. |
[20] | [Online]. Available: https://www.watertechonline.com/wastewater/article/15547507/understanding-ultrafiltration. |
[21] | [Online]. Available: https://www.waterfiltermag.com/water-filters/type/nanofiltration/. |
[22] | [Online]. Available: https://www.lenntech.com/processes/uhrpo.htm. |
[23] | [Online]. Available: https://www.danfoss.com/en/products/hpp/energy-recovery-devices/energy-recovery-device-for-small-to-medium-swro-applications/#tab-overview. |
[24] | [Online]. Available: https://www.watertechonline.com/home/article/14171280/membrane-cleaning-design-and-operation-of-ro-system-clean-in-place-skid. |
[25] | [Online]. Available: https://www.wateronline.com/doc/advanced-controls-microfiltration-0001. |
[26] | [Online]. Available: https://www.waterworld.com/filtration/article/14166930/turbidity-measurement-in-mf-systems. |
[27] | [Online]. Available: https://www.semanticscholar.org/paper/Ultrafiltration-Membrane-Technology-for-Water-Cleaning-Burgess/7a0d40f4e4d11bd84c2ac8b143c9e4fca66d8b28. |
[28] | [Online]. Available: https://www.watertechonline.com/advanced-controls-for-reverse-osmosis/article/14166573/advanced-controls-for-nanofiltration-systems. |
[29] | [Online]. Available: https://www.waterworld.com/filtration/article/14166573/advanced-controls-for-reverse-osmosis. |
[30] | [Online]. Available: https://www.semanticscholar.org/paper/Control-Strategies-for-Reverse-Osmosis-Water-Smith-Knapp/e41c8f826034b65f25e6ae8a7a65ea2c5a17a77d. |
[31] | [Online]. Available: https://www.sciencedirect.com/topics/earth-and-planetary-sciences/microfiltration. |
[32] | [Online]. Available: https://www.sciencedirect.com/topics/earth-and-planetary-sciences/water-softening. |